Hydrogels versus Scaffolds: When to Choose What?
The 2D Culture Paradigm
Our collective understanding of various cell-based processes is primarily derived from experiments performed on flat, unphysiologically stiff materials such as polystyrene and glass. Although the simplicity of these platforms is enticing, cells cultured under these circumstances tend to deviate from native cell behavior due to flattened shape, aberrant polarization, altered response to pharmaceutical agents, and loss of differentiated phenotype.
While these culture systems constitute a two-dimensional environment, the cells in the human body are more likely to receive signals not only at their ventral surface, but in all three dimensions. In addition, the cellular microenvironment often plays a significant role in disease progression and cellular responses to drugs. Therefore, culture systems that more effectively mimic the native biological environment are required to bridge the gap between conventional cell cultures and complex innate in vivo environments (1, 6).
To Scaffold, or Not to Scaffold
In modern research, a dichotomy of somewhat opposing methods is emerging; porous, structural scaffolds versus elastic, synthetic hydrogels. Both methods enable the creation of three-dimensional microphysiological systems (MPS) and thus carry the potential to more accurately mimic in vivo environments compared to 2D cultures. Each method presents their respective scientific advantages and limitations in recapitulating the properties of native tissues and is thus suitable for mimicking different types of tissues or in vivo environments. In consequence, to bridge this dichotomy, a distinction between when each method is more appropriate is necessary.
Hydrogels
Hydrogels are three-dimensional cross-linked polymer networks, which can absorb and retain large amounts of liquid. In fact, the first hydrogel material emerged in literature during the 1960s, when Wichterle and Lim reported a biocompatible hydrogel used for permanent contact with human tissues (e.g., contact lenses and arteries). Various functions of the native extra-cellular matrix (ECM), such as structural integrity, elasticity, biocompatibility, and bioactivity, can be effectively replicated in hydrogels. Due to their highly tunable properties and functionalities, as well as their relatively simple fabrication process, these gel-like constructs can be inherently beneficial for 3D cell culturing, being capable of mimicking the biochemical and mechanical properties of the native ECM (Fig. 1) (2).
Yet, despite constituting a major potential in biomedical applications and tissue engineering, hydrogel-based approaches also encounter substantial challenges. Hydrogels (such as those of collagen) tend to lack reproducibility, namely natural hydrogels have inherent batch-to-batch variability, thereby causing variations in the environment in which your cells grow. Moreover, the closed environments offered by hydrogels present limited possibility for long term culturing and repeat dosing. They also carry an increased risk of nutrient deficiency and hypoxia in their core, as well as uneven distribution of nutrients. Finally, hydrogels present some practical limitations, including risky handling as hydrogels can easily be lost during media changes and tend to peel off the dish during extended culturing (17).
Scaffolds
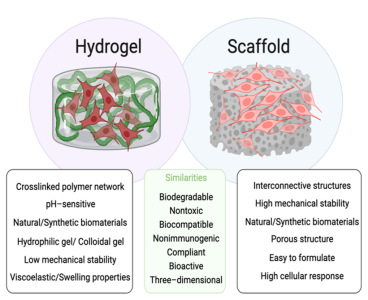
Figure 1: Schematic representation of structure, differences, and similarities associated with hydrogels and scaffolds.
Like hydrogels, a wide variety of cells and microorganisms can be seeded and cultured on scaffolds. Scaffolds can act as templates that mimic the functions of the ECM, allowing cells to interact and differentiate into their native phenotype. Furthermore, scaffolds exhibit functions that are essential to the success of tissue regeneration. These functions include providing a temporary support structure for tissue to be replaced; serving as a substrate for cell growth, proliferation, migration, and differentiation, providing spaces for vascularization and the formation of new tissues and their remodeling; and allowing for efficient transport of nutrients and growth factors (3).
Furthermore, porous scaffolds can provide a high degree of customization to directly influence cell patterning and conveying biochemical signals in a temporally and spatially controlled manner (Fig. 1) (4). In fact, since scaffolds can be produced – even mass customized – with no batch-to-batch variability, it is easy to ensure reproducibility and controllability when using scaffolds.
Scaffolds effectively support the assembly of cells and allow them to expand to significant numbers, providing an open environment with plenty of cell growth sites that allows for long term cell growth and repeat dosing (Ossiform has had a scaffolds-based culture of human mesenchymal stem cells going for 16 months). Finally, these constructs provide an easy-to-use interface with very low risk of losing your culture during media changes or rough handling of plates. Meanwhile, by default, scaffolds do generally not contain bioactive molecules, such as growth factors or ECM proteins, which can be key for truly mimicking the ECM. Instead, such molecules must be added exogenously. Furthermore, it is important to note that the material used for constructing the scaffold can significantly impact cell adhesion, growth, and behavior. It can therefore be highly beneficial to use materials that are endogenous to the human body, such as calcium phosphate.
Static and Dynamic In Vitro Mimetic Models
Whether scaffold-based or scaffold-free, in vitro mimetic models may be static or dynamic. Static in vitro models refer to assays where the cells are allowed to settle on 2D, or 3D adhesive substrates cultured with no flow and no mechanical stimulation. These models are widely used due to their easy-to-use interface and provide high throughput screening. Consequently, they can fail to mimic dynamic in vivo conditions of the human body, with the risk of generating ambiguous results with regards to specific cellular receptors, growth factor release, gene- and protein expression, and even proliferation, viability, and differentiation (6). On the other hand, dynamic in vitro models aim to provide an environment similar to in vivo conditions characterized by a continuous supply of oxygen and nutrients, as well as mechanobiological conditions including flow and pressures.
The Rise of In Vitro Models: Soft and Hard Tissues
Though in vivo models are still considered the gold standard for investigating diseases and developing novel therapies – and thus constitute a central component in pre-clinical research – animal models do not accurately represent the native human condition and display uncontrollable variables that result in experimental inaccuracy. Moreover, due to ethical concerns related to bestial discomfort and distress, their use should aim to align with the philosophy of the 3Rs – to reduce, refine, and replace animal testing. Collectively, these limitations of in vivo models give rise to the development of new in vitro alternatives.
In fact, much progress has been made within the field of microphysiological systems (MPS) in recent years. Today, in vitro models of various organs and tissues are commercially available, including, but not limited to, kidney, heart, neuro, liver, lung, cartilage, skin, gut, and vascular models (5, 6). As previously established, the features of the scaffold or hydrogel determine its fitness for the study or application. The material’s scale and structure, chemical and mechanical properties, matrix stiffness, and permeability can all significantly impact cell adhesion, growth, and behavior.
In this context, up until today, the focus of MPS developments has mainly revolved around mimicking soft tissue organs, for which hydrogels have proved highly suitable. Meanwhile one vital structure has been overlooked – that is, our bones.
Scaffolds as In Vitro Models for Studying Bone Remodeling
As nature teaches, bone is a connective hard tissue constituted by the extra-cellular matrix (ECM), collagen, bone apatite, osteocytes, and osteoblasts which control and adapt the structure performance based on mechano-sensitivity processes (7). In this context, the use of ceramic scaffolds for establishing in vitro bone models is promising because they can achieve an ideal rigidness and stiffness for emulating the native bone tissue environment as well as the high calcium concentration found in the mineralized part of human bone.
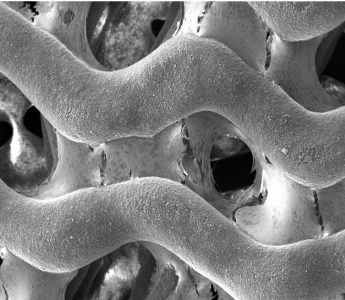
P3D Scaffolds are made from pure β-TCP. The image shows how the P3D Scaffold allows osteoblasts to produce an ECM that spans throughout the scaffold in an in vivo like manner.
Historically, rodents have been popular for predicting bone disorders due to the relatively minimal public opposition to their use, as well as low cost and ease of housing in comparison to other, larger animal alternatives. (8). Yet, with the emergence of new technologies, the use of scaffolds for creating in vitro bone MPS may now help improve our understanding of the molecular mechanisms and biological factors by which our bones remodel.
P3D Scaffolds are made from pure β-TCP. The image shows how the P3D Scaffold allows osteoblasts to produce an ECM that spans throughout the scaffold in an in vivo like manner.
Even the simplest in vitro model for bone remodeling fundamentally requires the co-culture of osteoblasts and osteoclasts. These can be performed either indirectly or directly: indirect methods comprise conditioned media, where media from one cell type is transferred to another, and the use of transwell inserts which provide two culture surfaces in the same well, allowing exchange of soluble factors but no cell-to-cell contact between the two types. On the other hand, direct methods co-culture both cell types on the same surface – be it a planar 2D tissue culture well or a 3D scaffold. For co-culturing, 3D scaffolds pose the advantage that they allow cells to expand to significant numbers and promote crosstalk between cells, thereby enabling long term tunable cultures where cells and other components may be added at different stages.
Towards a Sustainable Future
Both scaffolds and hydrogels have gained substantial attention for their potential to mimic various human in vivo situations and ultimately enhance our understanding of the physiological, pathological, and regenerative processes that occur in the human body. Yet, their potential extends far beyond.
Recently, the increasing global demand for energy and clean water has promoted researchers to develop sustainable energy and water technologies (9). Such technologies support the Sustainable Development Goals (SDGs) that were established by the United Nations General Assembly in September 2015. Especially, the SDGs concerning sustainable consumption and marine biomass, can be significantly influenced by this interdisciplinary field. In alignment with the SDGs, hydrogels have been integrated into a sustainable agriculture irrigation system (10), posed a potential alternative to plastic (11), and even cultivated to generate synthetic cellulose to promote a new generation of sustainable plants (12). By the same token, scaffolds have been successfully implemented for stem-cell based cartilage tissue engineering (13), as biomaterials for regenerative medicine (14, 15), and platforms in wound healing (16).
Not least, scaffolds and hydrogel technologies contribute to the rise in initiatives that can replace, reduce and refine animal testing. With new regulations in both the EU (Directive 2010/63/EU)
and USA (The Modernization Act 2.0), preclinical research too is aiming toward a more sustainable future. That is, a future in which animal research can be exhaustively reduced through the advancement, recognition and spread of non-animal testing strategies.
References
- Caliari SR, Burdick JA. (2016) A practical guide to hydrogels for cell culture. Nat Methods.
- Wang W., Narain R., Zeng H. (2020). Chapter 10 – Hydrogels. Polymer Science and Nanotechnology.
- Karande, T.S., Agrawal, C.M., (2008). Functions and requirements of synthetic scaffolds in tissue engineering. Nanotechnol. Tissue Eng. Scaffold.
- Hofer M., Lutolf M.P. (2021). Engineering organoids. Nat. Rev. Mater.
- The 3Rs Collaborative: https://www.na3rsc.org/microphysiological-systems-companies/
- Elliott NT, Yuan F (2011) A review of three-dimensional in vitro tissue models for drug discovery and transport studies. J Pharm Sci.
- Fung, Y.C. (1993). Biomechanics. Springer-Verlag, New York.
- Owen R and Reilly GC (2018) In vitro Models of Bone Remodelling and Associated Disorders. Front. Bioeng. Biotechnol.
- Guo Y, et al. (2020).Chem Rev.
- Mondal, M.I.H., Haque, M.O. (2019). Cellulosic Hydrogels: A Greener Solution of Sustainability. In: Mondal, M. (eds) Cellulose-Based Superabsorbent Hydrogels. Polymers and Polymeric Composites: A Reference Series. Springer, Cham.
- Wang, J., et al. (2019). Plastic-like Hydrogels with Reversible Conversion of Elasticity and Plasticity and Tunable Mechanical Properties. ACS applied materials & interfaces, 11(44).
- Chen C, Xi Y, Weng Y. (2022). Recent Advances in Cellulose-Based Hydrogels for Tissue Engineering Applications.Polymers.
- Zhao X, et al. (2021). Applications of Biocompatible Scaffold Materials in Stem Cell-Based Cartilage Tissue Engineering. Front Bioeng Biotechnol.
- Thygesen, T., et al. (2022). Comparison of off-the-shelf β-tricalcium phosphate implants with novel resorbable 3D printed implants in mandible ramus of pigs. Bone.
- Mazzoni E, et al. (2021). Bioactive Materials for Soft Tissue Repair. Front Bioeng Biotechnol.
- Rodríguez-Cabello JC, et al. (2018). Bioactive scaffolds based on elastin-like materials for wound healing. Adv Drug Deliv Rev.
- Habanjar O, et al. (2021). 3D Cell Culture Systems: Tumor Application, Advantages, and Disadvantages. Int J Mol Sci.