Why 3D bone printing is a foundation for pioneering research
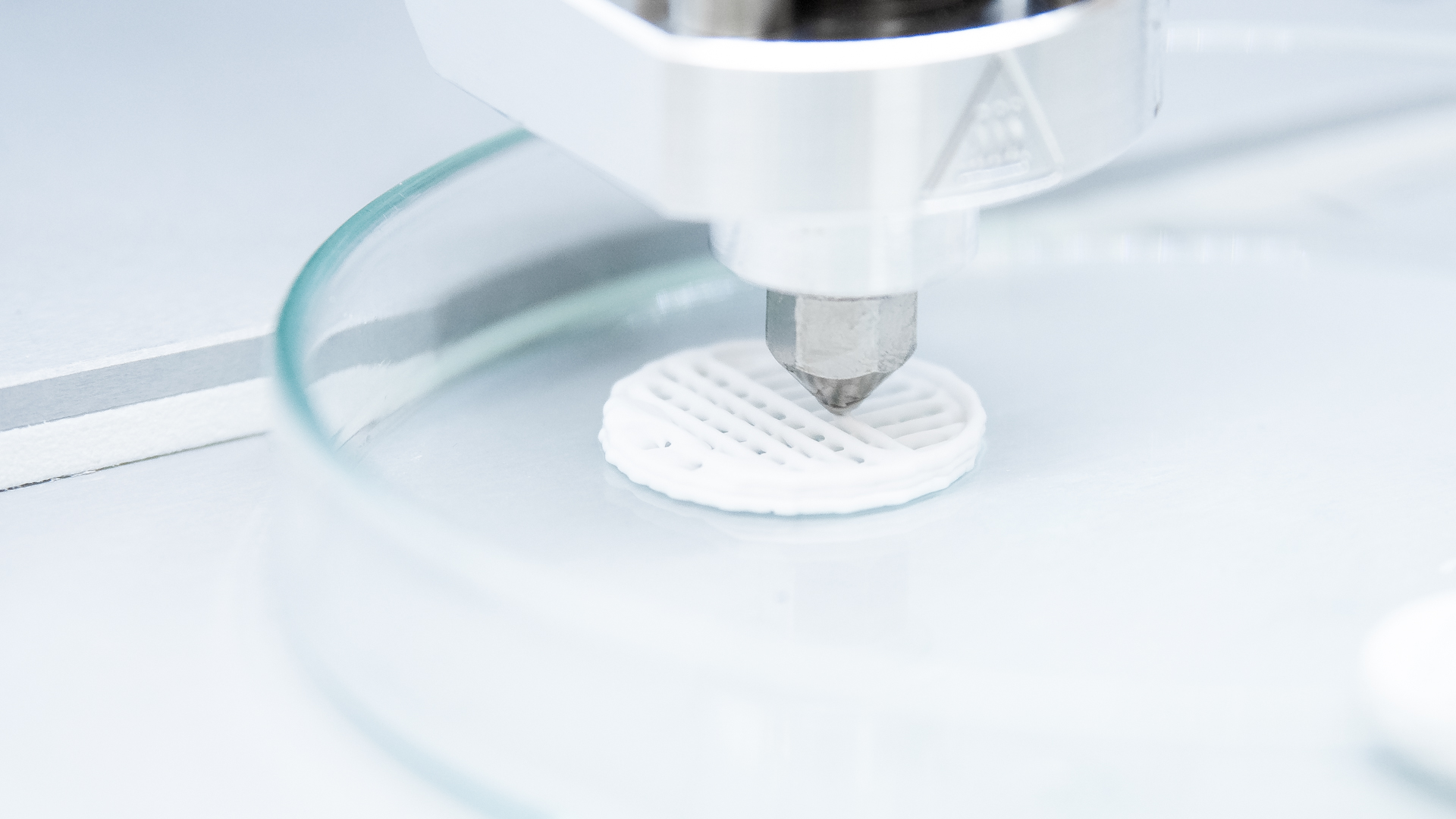
Why 3D bone printing is a foundation for pioneering research
Transformative research is unpredictable, and the pioneers of such research may not always realize when they are on the cusp of a radical breakthrough. Many research pioneers begin their careers with an insatiable curiosity and drive to address unmet challenges. Indeed, Emerson once said, “Do not go where the path may lead; go where there is no path and leave a trail.”
However, establishing a completely new field or path can be risky. Curiosity-driven, high-risk, high-reward research paths do not guarantee a hit research paper or a tenure-track position. Scientists, especially early-career stage researchers, and their groups must balance low and high-risk projects as they navigate the ‘publish or perish’ culture.
Yet, it may be comforting to acknowledge that transformative findings and breakthroughs are rarely stand-alone events (Gravem et al., 2017). Instead, they are shaped by social, economic, and even remote technological developments, such as 3D additive manufacturing.
Consider for a moment there was a time when radical treatment of bone and joint injuries and diseases was amputation (Gomez et al., 2005). In the pre-anesthesia era of the 1700s and 1800s, the rudimentary procedure was valued for its quickness. From this day forward, however, transformative and state-of-the-art treatment will involve the addition and proliferation rather than the excision of bone.
3D bone printing is laying the foundation for transformative research
If transformative research is built upon incremental advancements, one only needs to review the current capabilities of 3D bone printing and biocompatible ink. Consider the Nobel-winning gene-editing tool (CRISPR-Cas9) that was published rather quietly in 2012 (Jinek et al., 2012). Today, it’s one of the most highly cited and influential procedures. The ‘molecular scissors’ capabilities accelerated research in genetic diseases, agriculture, and drug development.
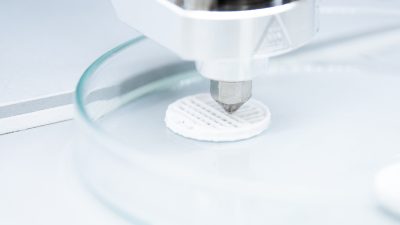
Image 1: Ossi Ink being printed on 3D printer
In another context, replacing the hip joint with an artificial one was once only conceptual. At least until a medical professor, Sir John Charnley, designed and performed the first truly long-lasting replacement in 1962. Since then, the orthopedic procedure (total hip arthroplasty or THA) has been the most implemented worldwide. THA and then eventually total knee arthroplasty (TKA) catalyzed an intense and massive research field in its own right (Holzer and Holzer, 2014). The field has become so large that it has already begun evaluating the benefits of 3D bone printing to help prepare for more complex cases of THA (Anzillotti et al., 2024).
Where will we see bone scaffolds give way to innovation?
In the case of 3D bone printing and ease of use, we expect to see an acceleration of established research paths and the creation of new ones. Both will be underscored by the advantages of experimenting with structures (scaffolds) that mimic the physical properties of natural bone in vitro.
While 2D cell cultures are affordable and straightforward, allowing the study of how bone cells interact, they cannot mimic the complex 3D environment of real bones. Research groups breaking free from traditional petri dish experiments to test their hypotheses stand to benefit the most. That is, the results are more likely to endure a direct in-vitro to in-vivo translation.
Generally, there is a major need to test, develop and improve in-vitro experimental conditions. When that need is met, it can lead to innovative results like the recently developed bone-on-chip (BoC) device. Coupled with the capabilities of 3D bone printing, the BoC device is designed to understand the remodeling process from start to finish (Nasello et al., 2021; Arrigoni et al., 2017). Similar to working with bone scaffolds alone, the BoC device can extend testing drug efficacy and side effects on bone activity, potentially shortening the time to reach therapeutic success.
As of now, new procedures and applications are ripe for discovery. To list a few of them:
- Orthopedics and related surgical fields will see immediate gains from substitutes for implantable bone, addressing bone defects, fractures, and trauma (Jensen et al., 2020; Tharakan et al. 2020).
- Oncology and immunology will see new routes to deliver osteogenic and anti-bacterial treatment alongside personalized therapeutics (Jensen et al., 2018).
- Biomedical and tissue engineering will see new possibilities to build functional structures, organs, and the next generation of biosensors (Lam et al., 2023).
3D bone-printed scaffolds can reduce experimental risks
The technology level of 3D bioprinters is generally at a point of maturity where research teams can affordably adopt higher-risk and higher-reward projects. At the same time, 3D bone-printed scaffolds eliminate specific risks that would normally weaken translational findings in traditional 2D cell cultures. 3D bone-printing outshines 2D cell cultures by significantly improving:
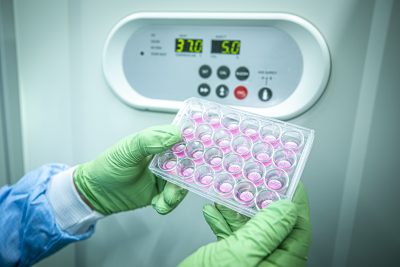
Image 2: Full plate of P3D Scaffolds
- Reproducibility
- Stability
- Batch-to-batch variance
Still, there are more direct benefits of 3D bone-printing that will underpin transformative research. Part of that transformation will start by replacing the needs and costs and removing the risks of ‘translational failure’ associated with using animal models. That is, the results obtained using animal models often fail to translate in the same way to humans. It is well understood that, for example, drug development is hindered by low animal-to-human success rates (Leenaars et al., 2019) and 3D bone-printing is uniquely positioned to change those statistics.
Success is achieved with biocompatible ink formulations
In addition to printing technologies, transformative research will heavily depend on the compositions of the biocompatible inks (Verma et al., 2023) used to print the scaffolds. These biomaterials are generally classified into two types: natural and synthetic. Each type has unique characteristics, such as how well they can be printed and their ability to support cell adhesion and growth over time. Also, for a research model, the scaffold must make it easy to identify the material deposited by osteoblasts.
Amongst the most critical parameters for a printed scaffold, as shown for Ossiforms’ β-TCP, or beta-tricalcium phosphate formulation, is that it is osteoconductive, resorbable in vivo yet stable in vitro, provides relevant biomechanical strength and stiffness, and does not elicit an immune response (Thygesen et al., 2022).
How to get 3D bone printing into the laboratory
Print-on-demand is set to become the new gold standard, with access to 3D bone printing emerging as a hallmark of cutting-edge research and laboratories. At Ossiform, we know that the path to innovation needs the backing of research grants, and 3D bone printing can provide a competitive edge. Feel free to contact us for:
- Expert guidance on how to begin using 3D bone printing in your laboratory.
- Recommendations for protocol development and assistance.
- Help with grant quotes and advice on budgeting.
- More information about our scaffolds and biocompatible inks.
References
- Anzillotti, G. et. al. (2024). Using Three-Dimensional Printing Technology to Solve Complex Primary Total Hip Arthroplasty Cases: Do We Really Need Custom-Made Guides and Templates? A Critical Systematic Review on the Available Evidence. Journal of Clinical Medicine, 13(2), 474.
- Arrigoni, C. et. al. M. (2017). Bioprinting and organ-on-chip applications towards personalized medicine for bone diseases. Stem cell reviews and reports, 13, 407-417.
- Gomez, P. F., & Morcuende, J. A. (2005). Early Attempts at Hip Arthroplasty: 1700s to 1950s. The Iowa Orthopaedic Journal, 25, 25-29.
- Gravem SA, et. al. Transformative Research Is Not Easily Predicted. Trends Ecol Evol. 2017 Nov;32(11):825-834. doi: 10.1016/j.tree.2017.08.012. Epub 2017 Sep 15. PMID: 28923494.
- Jensen, M. B. et. al. (2020). Treating mouse skull defects with 3D-printed fatty acid and tricalcium phosphate implants. Journal of Tissue Engineering and Regenerative Medicine, 14(12), 1858-1868.
- Jensen, M. et. al. (2018). Composites of fatty acids and ceramic powders are versatile biomaterials for personalized implants and controlled release of pharmaceuticals. Bioprinting, 10, e00027.
- Jinek, M. et. al. (2012). A Programmable Dual-RNA–Guided DNA Endonuclease in Adaptive Bacterial Immunity. Science.
- Holzer, L. A., & Holzer, G. (2014). The 50 Highest Cited Papers in Hip and Knee Arthroplasty. The Journal of Arthroplasty, 29(3), 453-457.
- Huang, H. et. al. (2023). 3D-printed tri-element-doped hydroxyapatite/ polycaprolactone composite scaffolds with antibacterial potential for osteosarcoma therapy and bone regeneration. Bioactive Materials, 31, 18-37.
- Lam, E.H., Yu, F., Zhu, S., & Wang, Z. (2023). 3D Bioprinting for Next-Generation Personalized Medicine. International Journal of Molecular Sciences, 24.
- Leenaars, C. et. al. (2019). Animal to human translation: a systematic scoping review of reported concordance rates. Journal of Translational Medicine, 17(1).
- Nasello, G., Cóndor, M., Vaughan, T., & Schiavi, J. (2020). Designing Hydrogel-Based Bone-On-Chips for Personalized Medicine. Applied Sciences, 11(10), 4495.
- Tharakan, S., Khondkar, S., & Ilyas, A. (2020). Bioprinting of Stem Cells in Multimaterial Scaffolds and Their Applications in Bone Tissue Engineering. Sensors, 21(22), 7477.
- Thygesen, T., et. al. (2022). Comparison of off-the-shelf β-tricalcium phosphate implants with novel resorbable 3D printed implants in mandible ramus of pigs. Bone, 159, 116370.
- Verma, S., Khanna, V., Kumar, S., & Kumar, S. (2023). The Art of Building Living Tissues: Exploring the Frontiers of Biofabrication with 3D Bioprinting. ACS Omega.