Democratizing 3D Bone Printing for Medical Innovations
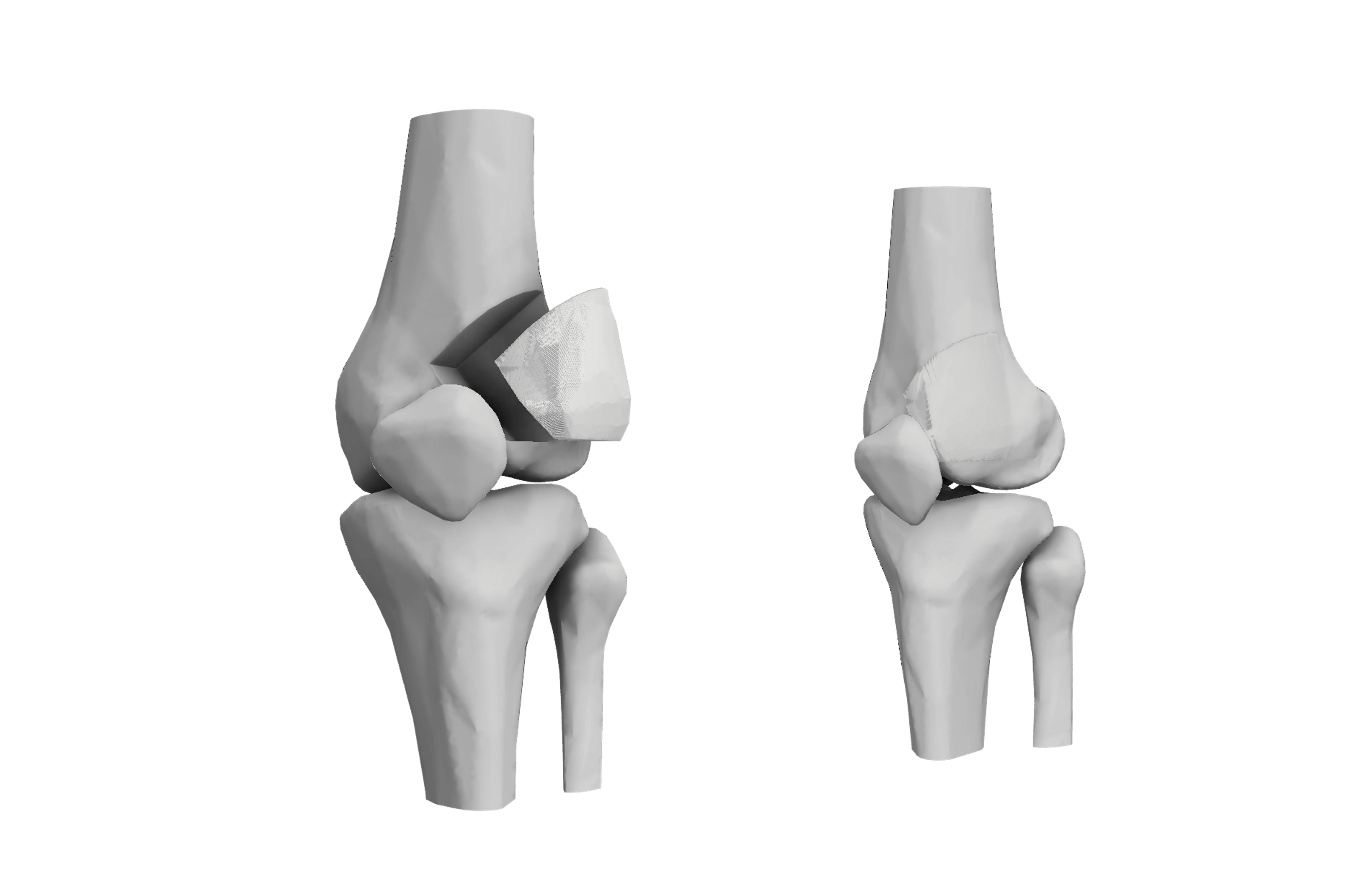
Democratizing 3D Bone Printing for Medical Innovations
The democratization of 3D bone printing
The democratization of advanced technology, particularly in 3D bone printing, represents a shift towards harnessing global expertise rather than engaging a select few to tackle pressing medical challenges. Previously, only some medical professionals, researchers, and institutions could raise enough funds to use the latest medical technology in their research. But Ossiform’s 3D bone printing services are changing that narrative.
3D bone printing is paving the way for more equitable and affordable access for researchers, especially those aspiring to develop and innovate in orthopedics and oncology. What’s more, removing economic barriers enables the mobilization of a broader research community.
Equipping a larger community of researchers and clinicians with 3D bone printing will accelerate collective intelligence and collaboration opportunities, increasing the likelihood of producing high-impact research, patents, and medical innovations (Uzzi et al., 2013; Mukherjee et al., 2017). Yet, getting there will take some time and effort.
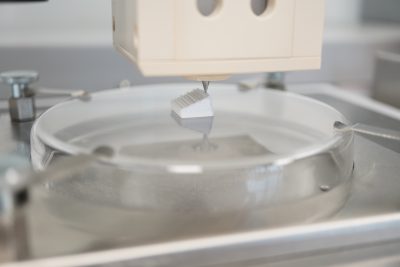
Image 1: Implant being printed with 3D printer
How likely will 3D bone printing become mainstream?
In the late 1990s and early 2000s, 3D printing technology primarily focused on industrial uses.
By the late 2000s, the marked decrease in the cost of 3D printers made them more accessible to a broader audience. The result is that 3D printing now supplies customizable and rapid delivery of parts for jet engines, medical devices, home appliances and more (Daly, 2015).
So, if history offers any insight, medical grade 3D printed bone implants will soon be delivered to surgery wards. This means bone scaffolds will be sent to the laboratory to test the latest cancer therapy. And all this scientific progress will be made possible by democratizing 3D bone printing.
Scientific progress in 3D bone printing
The first article citing 3D bone printing within bone tissue engineering was published in 2004; three more were published in 2005. As we approach hundreds today, the field is reaching a tipping point where scientific progress will combine the advantages of 3D bone printing and the wealth of existing scientific knowledge.
One of the most innovative uses of 3D printing is making implants to treat extensive (>5 cm3) bone loss and trauma. There is a consensus that autologous bone grafts have several practical limitations. First, there is often a limited amount of available bone a patient can provide for grafting; in addition, there are post-surgical complications, such as pain, slow healing time, and donor site morbidity.
Although it’s still in the early days, with limited clinical data, the initial outcomes of tissue engineering, made possible by 3D bone printing, are promising.
Rebuilding significant bone gaps and defects, especially those as big as 12 cm3, is an unmet clinical need. To tackle this, researchers are testing in vivo models that closely mimic human conditions. In one case, researchers implanted a customized 3D PLA implant coated with an osteogenic bioactive molecule called bone morphogenetic protein 2 or BMP-2 directly into a bone area needing repair.
Adding stem cells to implants
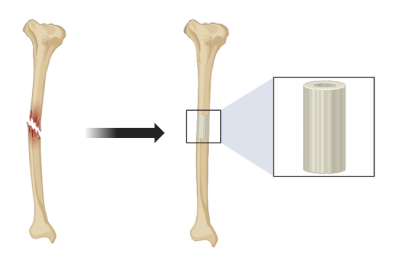
Image 2: Repair of bone defect with 3D printed implant
Stem cells can also be added to the implant to enhance bone growth. However, doing so involves a sophisticated setup of harvesting and expanding them in culture before placing the implant. This is why 3D bone-like printed scaffolds for research need a material that can promote cell adhesion and growth. As an option for customized implants, research groups are now exploring the clinical utility of recruiting the patient’s own stem cells in vivo. Coating the 3D-printed PLA implants with growth factors, such as BMP-2, signals nearby stem cells, which then appear to migrate to the implant site.
The team compared how well the 3D-printed bone-mimicking implant worked against the traditional method of using autologous bone grafts. The authors found significant bone growth and faster healing and credited the success of carefully dosing the scaffold with BMP-2. New bone grew evenly within the scaffold, underscoring the promise of rebuilding critical-sized bone defects in patients using 3D-printed scaffolds (Bouyer et al., 2021). What’s more, 3D-printed scaffolds can be personalized and made from natural, bone-like materials to fit each patient’s unique need. This is offering a better surgical environment and addressing anatomical and disease-specific challenges.
How democratizing 3D bone printing will lead to the ideal scaffold
Extensive research is still required to address several disease-specific challenges and ensure that the fabrication of 3D bone-printed scaffolds leads to desired patient outcomes. While there is an unmet clinical need for repairing bone defects due to trauma or cancer, which 3D bone-printed scaffolds can address, the ideal scaffold, per disease and patient condition, is relatively underexplored. Thus, democratizing 3D bone printing so that it is affordable and accessible is not only a feel-good initiative but also a needed one.
Traditionally, in orthopedics, β-tricalcium phosphate (β-TCP) is a frequently utilized and highly effective bioceramic material for bone implants (Liu et al., 2012). Its effectiveness stems from its ability to promote osteoblasts’ attachment, growth, and differentiation. This process is facilitated through a cellular signaling pathway triggered by the release of calcium and phosphate ions, essential minerals for forming new bone (Chen et al., 2015). This is also why Ossiform uses a nonpolymeric bioink consisting of β-TCP as a choice material for laying the foundation of 3D bone-like printed scaffolds.
What is the ‘ideal’ scaffold?
Arriving at conclusions that outline the properties of an ideal scaffold will likely start from incremental yet critical experimentation on smaller 3D-printed human-like bone scaffolds suitable for the petri dish rather than fully implantable 3D bone-printed constructs.
For example, researchers showed that trabecular-inspired patterned 3D bone-like scaffolds cultured with MC3T3 cells with, rather than without, the osteo-inductive agent Calcitonin Receptor Fragment Peptide (CRFP) improved the mechanical properties (Helguero et al., 2017). Both compressive strength and modulus increased, providing encouraging results that one day, printing entire bones, not just segments, for repairing critical-sized bone defects will be achievable.
Drawing from this, researchers will need to explore not one but many properties that help to define and refine the ideal scaffold designs by diving into:
- osteoconductive properties
- cell functionality and morphology
- mechanical stability
- remodeling potential
- reabsorption profile
- ability to promote vascular ingrowth
- performance of osteoinductive factors or cells
Ossiform aims to bring 3D bone printing to labs worldwide
There is plenty of uncharted ground for pioneering research, and using the cellular microenvironment mimicking human bone will undoubtedly lead to significant discoveries. Yet, current utilization is often constrained by the need for specialized equipment and high startup costs with 3D bone printing (D’Alessio et al., 2018). But today, that is no longer the case.
With mounting pressure to attract significant funds and less and less time to do so, Ossiform aims to democratize 3D bone printing by reducing the barriers to getting started. That means making medical technology and solutions equally accessible and more affordable, freeing up time to focus on critical questions that will lead to the next breakthrough.
Feel free to contact us to hear about the first steps your research team can take to get started.
References
- Chen, Y., Wang, J., Zhu, X., Tang, Z., Yang, X., Tan, Y. F., … & Zhang, X. (2015). Enhanced effect of β-tricalcium phosphate phase on neovascularization of porous calcium phosphate ceramics: in vitro and in vivo evidence. Acta Biomaterialia, 11, 435-448. https://doi.org/10.1016/j.actbio.2014.09.028
- D’Alessio, J., & Christensen, A. (2018). 3D Printing for Commercial Orthopedic Applications: Advances and Challenges. 3D Printing in Orthopaedic Surgery, 65-83. https://doi.org/10.1016/B978-0-323-58118-9.00007-5
- Daly, A. (2015). Regulating Revolution: An Introduction to 3D Printing and the Law. In Socio-Legal Aspects of the 3D Printing Revolution (pp. 1–17). Palgrave Macmillan UK. https://doi.org/10.1057/978-1-137-51556-8_1
- Haleem, A., Javaid, M., Khan, R. H., & Suman, R. (2020). 3D printing applications in bone tissue engineering. Journal of Clinical Orthopaedics and Trauma, 11(Suppl 1), S118. https://doi.org/10.1016/j.jcot.2019.12.002
- Helguero, C., Mustahsan, V., Parmar, S., Pentyala, S., Pfail, J., Kao, I., … & Pentyala, S. (2017). Biomechanical properties of 3d-printed bone scaffolds are improved by treatment with crfp. Journal of Orthopaedic Surgery and Research, 12(1). https://doi.org/10.1186/s13018-017-0700-2
- Liu, B. and Lun, D. (2012). Current application of β‐tricalcium phosphate composites in orthopaedics. Orthopaedic Surgery, 4(3), 139-144. https://doi.org/10.1111/j.1757-7861.2012.00189.x
- Mukherjee, S., Romero, D. M., Jones, B., & Uzzi, B. (2017). The nearly universal link between the age of past knowledge and tomorrow’s breakthroughs in science and technology: The hotspot. Science Advances. https://doi.org/1601315
- Uzzi, B., Mukherjee, S., Stringer, M., & Jones, B. (2013). Atypical Combinations and Scientific Impact. Science. https://doi.org/1240474